Blake M. Hofferber, Edward Kolodka, University of North Dakota, Grand Forks, ND, USA
Rishawn Brandon, Robert J. Moon, Charles R. Frihart
USDA Forest Products Laboratory, Madison, WI, USA; [email protected]
Introduction
The number of acceptable wood adhesives drops dramatically for applications in moisture-changing environments. Most research on wood-adhesive bond durability has considered surface adhesion as the critical factor for good bonds, focusing on the effects of adhesive-wood wetting, extractives, and surface preparation, for example. However, in moisture-changing environments, surface adhesion may not be the dominant factor in preventing bond failure. Dimensional strain in the interphase as wood moisture content (MC) changes may lead to sufficient bondline stress to cause fracture.
Because wood is a hygroscopic material, fluctuations in relative humidity (RH) cause considerable fluctuations in wood MC, resulting in appreciable dimensional changes. The anisotropic nature of the wood structure results in different mechanical properties and swelling– shrinking behavior in three distinct directions: longitudinal, radial, and tangential. Typically, maximum strain, as determined by drying from the green state to ~0% MC, is 0.1% to 0.3% in the longitudinal direction, 2.1% to 7.9% in the radial direction, and 4.7% to 12.7% in the tangential direction. Strain in the longitudinal direction is limited due to the predominant orientation of cellulose fibers in this direction [1,2].
Swelling–shrinking behavior of wood has been extensively studied [1-3], but relating this to stress and strain response along an adhesive bondline between two wood surfaces is difficult. In general, the amount of stress acting along the bondline will be influenced by several factors: swelling–shrinking coefficients of the wood, wood orientation on either side of the bondline, wood thickness, level of MC variation, rates of MC change, and viscoelastic properties of the wood and adhesive. Experimental measurement of stress distribution acting along a bondline is difficult; however, extensive studies on the swelling pressure of wood have indicated the amount of stress that can build up in the wood [3,4]. Bondline stress distributions have been estimated via laminate theory and finite elemental modeling and have given insights on minimizing bondline stresses for bonded wood assemblies [5,6]. However, predicted stress distributions are only as good as the estimates used in the model, such as mechanical property estimates, assumed moisture gradients, and ideal strain in wood.
Chemical treatments that increase the dimensional stability of wood in changing moisture environments can reduce the amount of differential swelling across the bondline, which is considered in this study to lower bondline stress. Acetylated wood has increased dimensional stability, resulting from its lower propensity for taking up moisture [7]. Acetylation is esterification of accessible hydroxyl groups in the cellulose and hemicellulose, where acetyl groups replace hydroxyl groups. Dimensional stability results from the reduction of active hydroxyl sites for water to bond with, which reduces fiber saturation point and equilibrium MC.
The purpose of this study was to investigate the role of wood swelling on performance of wood-adhesive bonds (resorcinol formaldehyde, epoxy, emulsion polymer-isocyanate), for untreated and acetylated wood. Effects of these treatments on measured strain anisotropy and swelling stress were measured and then related to compressive shear strength and percentage wood failure of wood-adhesive bonds.
Experimental
Yellow-poplar (Liriodendron tulipifera) sapwood samples, both untreated (control) and acetylated, were used in this study. Strain and stress measurements used cube block samples (25 by 25 by 25 mm) oriented such that faces were mainly transverse, tangential, and radial (Figure 1). The compression shear fracture experiments used samples 31 mm (longitudinal) by 25 mm (tangential) by 6 mm (radial). For strain and stress measurements, dry conditions refer to ~5% MC, whereas ~12% MC was used in fracture measurements. Wet conditions refer to “saturated” wood.
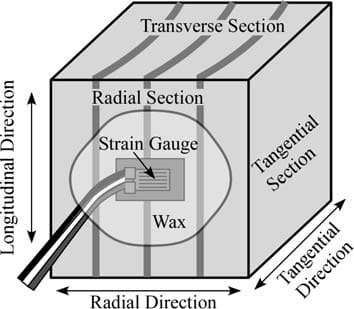
Figure 1. Schematic of strain gauge mounted on radial surface of wood sample, for measuring strain in the radial direction.
Strain Measurements
Strain measurements were completed using Vishay Micromeasurements CEA-06-125UN-350 option P2 strain gauge, which is accurate up to 5% maximum strain. Strain gauges were bonded to the wood surface at the center of a given cube face (Figure 1), and the samples remained in a desiccator at 33% RH and ~25°C until testing. Samples were placed in a beaker, and deionized water was added to the beaker until the upper surface of the cube was just covered by water. Strain measurements were made until the swelling strain plateaued, >5 h. Strains in tangential, radial, and longitudinal directions were measured.
Stress Measurements
Preliminary stress measurements were made using SensorProd Pressurex pressure-sensitive film, which contains microcapsules of a red die that rupture under a given applied stress. Degree of coloration is compared with a standard color-stress key, indicating the maximum pressure to which the film was subjected. Cube samples were placed in a confinement apparatus that provided triaxial restraint on wood swelling (Figure 2). In one orientation additional space allowed for placement of the pressure-sensitive film and a steel spacer plate; during sample confinement in the dry state, minimal pressure was placed on the film. The confinement apparatus was submersed in water for 72 h. Swelling pressures were measured resulting from strain in the following directions: control tangential and radial (10 samples each), acetylated tangential (6 samples), and radial (1 sample).
Fracture Measurements
Compression shear experiments of bonded wood samples were conducted according to ASTM D 905-98, and percentage wood failure assessment followed ASTM D 5266-99. Control and acetylated samples were conditioned at 27°C and 65% RH, and within 24 h of planing (control sample only), the samples were bonded. Three types of adhesives were investigated: resorcinol–formaldehyde (RF), epoxy, and emulsion polymer–isocyanate (EPI). Adhesives were spread at a rate of 320–340 g/m2 and cured at room temperature. Fracture measurements were conducted for both dry (27°C and 65% RH) and wet conditions. Samples were “saturated” by submerging in tap water at room temperature in a pressure vessel, applying a weak vacuum of 0.84 bar for 30 min, and then applying a pressure 4.48
bar for 30 min. Samples remained submerged in water until tested. A total of 216 samples (18 samples for each of 12 conditions) were tested.
Results and Discussion
Average maximum water absorption swelling strain (plateau value) for control and acetylated samples are given in Table 1, which shows the ~50% reduction in maximum strain for the acetylated wood.
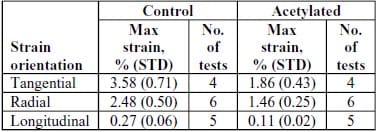
Table 1: Average Maximum Swelling Strain
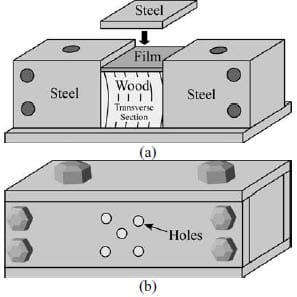
Figure 2. Schematic of swelling stress testing configuration. (a) Sample in confinement with transverse section positioned outward in this orientation, swelling pressure in tangential direction to be measured; (b) confinement on all sides of wood specimen, holes allow water to contact the transverse section of the wood specimen.
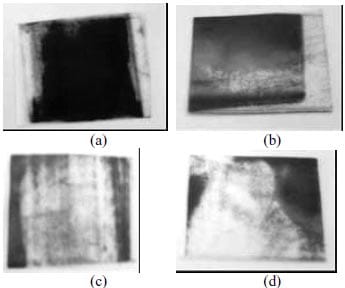
Figure 3. Post-tested pressure-sensitive films. Dark shading represents regions of higher stress. (a) Untreated tangential swelling, (b) untreated radial swelling, (c) acetylated tangential swelling, and (d) acetylated radial swelling.
The pressure-sensitive film placed between the wood and restraining surface measured stress exerted by the swelling wood against the restraining structure. For all samples tested, maximum swelling stress was ~9.7 MPa, independent of swelling direction or wood treatment. Figure 3 shows representative images of post-tested films. Stresses within the center of the acetylated samples appear to be much lower than those of the control samples.
Compressive shear strength and percentage wood failure results for control and acetylated samples tested in dry or wet condition are given in Table 2. For control samples, dry shear strength was ~12 MPa, with wood failure of 90% to 100%, and was nearly independent of the adhesive used. Under wet conditions, shear strength was noticeably lower, ~5 MPa. For RF and EPI adhesives, percentage wood failure was same for dry and wet conditions, suggesting that the decrease in shear strength resulted from wood plasticization due to water uptake.
Acetylation, under dry testing conditions, had a small effect on shear strength and on percentage wood failure. However, under wet conditions, acetylation influenced the resulting fracture properties, and it is considered here that the following factors may have been modified: wood-adhesive bond strength, wood plasticization, and swelling strains. For the RF adhesive, acetylated wood shear strength was 9.4 MPa, which is 1.7 times that of the control sample. Percentage wood failure was the same for control and acetylated wood, suggesting that changes in wood fracture properties are primary contributors to the increase in shear strength. This increase in strength was attributed to a reduction in wood plasticization resulting from acetylation of the wood.
For the epoxy case, reduction in shear strength in the wet condition is believed to result partially from wood swelling strain. Acetylated wood shear strength was 9.8 MPa, which is 2.1 times that of the control sample. By comparing results between control and acetylated samples, and considering that swelling strains in acetylated samples were ~50% lower than in the control samples, the influence of swelling strain may be inferred. For control samples, wood failure in the dry condition was 90% and became 0% in the wet condition; for the acetylated sample in the wet condition, wood failure was 60%. It is considered here that the smaller swelling strain in the acetylated sample induced a lower bondline stress. Assuming that bondline failure occurs once a critical stress is achieved, by reducing internal stress from wood swelling, the bondline can withstand a larger external shear stress before failure. Additionally, if the bondline is sufficiently strong, fracture will change from along the bondline to within the wood. However, additional variables must be considered before one can confidently state the extent to which swelling strain influences the resulting fracture behavior, such as water interactions with the adhesive, and the adhesive-wood bonding mechanism.
For EPI adhesive, acetylated wood shear strength was 3.3 MPa, which is 0.6 times that of the control sample. The complete reduction in percentage wood failure with acetylated wood under wet conditions shows that reduction in the adhesive-wood bond strength occurs, which may result from an interaction of water with the EPI–acetylated wood bonding sites.
Conclusions
This preliminary investigation has considered that swelling strains, as a result of changes in wood moisture content, lead to bondline stresses that modify the bondline fracture and influence bond durability. The differences in maximum swelling strain due to water absorption between untreated wood and acetylated wood were used to investigate the role of swelling strains on the fracture behavior of bonded assemblies. The swelling stress experiments demonstrated that yellow-poplar has sufficient swelling strength to strain an adhesive at a given bondline.
The influence of wood swelling strain on the measured shear strengths for the RF– and EPI–yellow-poplar adhesive systems was believed to be minor. However, for the epoxy–yellow-poplar adhesive system, the reduction in swelling strain for acetylated wood was believed to produce a lower bondline stress from dry to wet condition, resulting in larger measured shear strengths as compared with untreated wood. Thus, for environments that cause MC changes within the wood, bond durability of the ep-oxy–acetylated wood would be expected to be higher because of the reduction of maximum swelling strain and bondline stress.
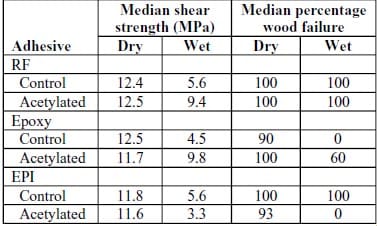
Table 2: Shear Strength and Percentage Wood Failure
References
- K. Kelsey, CISRO Aust., Div. For. Prod. Tech. Paper No. 28. 1963.
- S. Pang, Wood Sci. Tech., 2002, 36, pp 75-91.
- C. Skaar, Wood-Water Relations, 1988, Springer– Verlag, New York.
- T. Perkitny and R. S. T. Kingston, Wood Sci. Tech., 1972, 6, pp 215-229.
- T. Laufenberg, Forest Prod. J., 1982, 32(5), pp 42-48.
- A. G. H. Dietz, H. Grinsfelder, and E. Reissner. Transactions of the American Society of Mechanical Engineers, 1944, pp 329-335.
- E. Obataya and J. Gril, J. Wood Sci., 2005, 1, pp 124129.